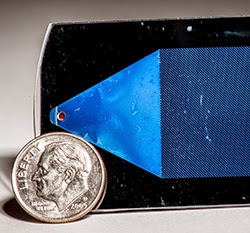
The impacts of biogeochemical processes in the underworld beneath our feet are on massive scales. Thousands of microbial species dine on organic molecules, belching their leftovers back into the soil and upwards into the atmosphere. Mineral-laden aquifers pulse with water that seasonally comingles with rivers rushing overhead. Fissures and faults in the earth provide conduits for subsurface chemicals to rise into the air. Each of these processes varies depending on the climate, the composition of minerals, and the tectonics of each region on the planet. Understanding what’s going on down there, and how it effects what’s going on up here, sounds like a herculean order. But at EMSL, scientists are getting a handle on these enormous macroscopic processes by zooming down to the microscopic scale.
By imprinting tiny replicas of sediment onto silicon wafers and employing a battery of imaging techniques to watch what unfolds as fluids pass through, scientists are observing the molecular processes that underpin massive ones, such as the carbon cycle and the transport of subsurface contaminants. EMSL scientists and users are interested in understanding all of these large processes and more, by examining them at the size that matters most: the pore scale.
Through creation of micron-scale models akin to tiny high-tech ant farms, and incorporation of supercomputer simulations that take diverse biogeochemical factors into account, researchers hope to connect what they learn at the molecular level with processes that affect our entire ecosystem.
“We’re starting with these molecular, pore-scale processes and tying them into the big earth-water system, and that’s crossing many, many scales,” says Nancy Hess, a Science Theme lead at EMSL.
Radioactive pores
The spread of subsurface contaminants depends largely upon whether they dissolve in mobile groundwater, or form precipitates that stay put. In the case of a uranium plume that lurks underground at the Hanford Site less than two miles from EMSL, scientists need to understand how fast contaminants will encroach on the nearby Columbia River. Even more pressing scenarios are playing out in contaminated uranium mining sites near waterways. The oxidation state of uranium is the main factor that dictates whether the contaminant becomes soluble (and therefore mobile), and the way uranium interacts with minerals dictates its oxidation state. Oxidized uranium (VI) is highly soluble, whereas its reduced cousin, uranium (IV), tends to precipitate.
Just measuring the chemical species present underground gives researchers a crude view of the complex interactions that dictate uranium precipitation, says Hess. In reality, steep geochemical gradients, consisting of mobile phases that flow through rock or percolate into it, create a dynamic mix of chemical species. The best way to truly understand these steep gradients and how they affect contaminants is to go down to the micron scale.
“We can create very steep geochemical gradients within these pore-scale models,” Hess says. “We’ve found that uranium may be in a very oxidizing environment during advective flow, but it can diffuse into a region that’s actually quite reducing. These reducing microenvironments lead to the trapping or sequestration of contaminants at a higher level than you would expect if you took this macroscopic view of the environment.”
Researchers can also use micromodels to predict how contaminants might respond to remediation efforts. For example, uranium can precipitate when it reacts with phosphate, so researchers have attempted to curb uranium mobility by amending the subsurface with phosphate. The process had been studied on larger scales – in bottles, core samples and in the field.
“What was missing was a fundamental understanding how groundwater flow, solute mixing and groundwater chemistry affect precipitation, and the form of the uranium phosphate that develops,” said Charles Werth of the University of Illinois at Urbana-Champaign. “We want to understand how groundwater conditions affect what’s forming, so we can then determine the potential for uranium remobilization in the future.”
Werth collaborated with EMSL researchers to build a micromodel and to study uranium transport and precipitation in response to phosphate addition. Much like fabrication of a microchip, the researchers used plasma etching to create a representative pore structure on a silicon wafer. Sealed with a glass coverslip and equipped with inlets and outlets, the micromodel allowed researchers to pump uranium, phosphate and other common groundwater constituents through the pore structure and view precipitation reactions in real time. The team used brightfield reflected microscopy to watch precipitates form, and employed Raman backscattering spectroscopy and micro X-ray diffraction to distinguish the mineralogy and chemical makeup of the precipitates.
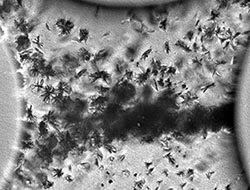
The researchers found uranium and phosphate precipitate into a mineral called chernikovite and this mineral rapidly clogs pores and reduces flow. The precipitation is a desirable effect; however, the dramatic pore blockage could limit the spread of phosphate underground, the team concluded.
“The advantage the micromodels give is they allow direct observation and spectroscopic interrogation of reactions occurring in a groundwater system,” Werth said. “The results give us insights into how effective that process might be at trapping uranium in the subsurface.”
The microbe factor
EMSL users also have their sights set on understanding how microscopic soil processes affect the carbon cycle. Plants remove carbon dioxide from the atmosphere, and later that carbon incorporates into the soil when the plants die. Microbes in the soil then digest the carbon and release it back into the atmosphere. Understanding the processes that affect microbial breakdown of carbon will have major impacts on models of climate change. As of now, the contribution of microbes to atmospheric carbon is a black box, says mineralogist Markus Kleber of Oregon State University in Corvallis. Kleber’s team plans to use EMSL’s expertise and instruments to understand how different minerals in the soil affect the ability of microbes to break down organic material.
Unlike humans and other animals, Kleber says, “microbes are tiny, and they have no mouth.” This seemingly obvious distinction makes a big difference in the way microbes digest carbon. Rather than processing their food internally, microbes must secrete enzymes into the surrounding soil to break down large organic molecules, such as cellulose. The microbes can then transport the smaller breakdown products across their membranes and utilize them for energy. This external digestion process means the secreted enzymes must carry out their functions amidst minerals in the soil.
Some mineral surfaces, such as manganese oxide and iron oxide, are considered “catalytic,” and may have catastrophic effects on the structure and, therefore, the function of microbial enzymes. “How do microbial enzymes interact with mineral surfaces?” Kleber wants to know. “Do they simply absorb, get a little dented, but otherwise stick around? Or do the enzymes come in contact with a very reactive mineral surface and get blown apart?”
To find out, Kleber and graduate student Stephany Chacon will collaborate with EMSL scientists to measure the structural changes that occur in proteins when they interact with various minerals. Using 2-dimensional nuclear magnetic resonance spectroscopy and Fourier transform ion cyclotron resonance, or FT-ICR, mass spectrometry, Kleber and Chacon will assess the structural changes that occur in a model protein when it interacts with manganese oxide and other reactive surfaces. The team will then mutate the protein to understand how various amino acid changes affect the way the protein survives mineral encounters. Kleber hopes the findings will eventually allow researchers to incorporate the known distribution of soil minerals on the planet into the Department of Energy-sponsored Community Land Model.
Permafrost secrets
No soil on the planet harbors as much subsurface carbon as does Arctic permafrost. Frozen underground for millennia, the carbon residing there is gradually breaking out of its icy cage as the planet warms, and soil microbes are awakening from their chilly slumber to reap the rewards. But the rate at which the microbes process the carbon and release it into the atmosphere depends on the composition of soil organic matter, which varies depending on the subsurface’s mineralogy. Getting a handle on the thousands of molecules that live in the soil has until recently been considered too daunting to attempt, according to Hess.
“The typical view of carbon in soil is that it’s a mess: just gunky molecules all glommed together like overcooked spaghetti,” says Hess. “Most scientists have just given up on trying to do any molecular characterization.” However, at EMSL, researchers are finding that through the use of high resolution techniques such as FT-ICR, the molecular secrets of Arctic permafrost carbon are finally being told.
As part of the DOE’s Office of Biological and Environmental Research-funded Next-Generation Ecosystem Experiments in the Arctic, which seeks to understand the decomposition rates and pathways of carbon in permafrost soils, scientists will use FT-ICR mass spectrometry to dissect the molecular makeup of samples extracted across the Arctic. Baohua Gu of Oak Ridge National Laboratory is heading the biogeochemistry effort on soil organic matter.
“By identifying and quantifying the key soil organic carbon precursors to microbial production of small organics and/or byproducts, we can elucidate degradation pathways and contributions of soil organic matter in greenhouse gas production under future climate scenarios,” says Gu.
He hopes the new information will lead to improved climate modeling, allowing for more accurate predictions on the feedback of greenhouse gases in a warming climate. Alongside EMSL scientists, Gu also hopes to learn how carbon release and changing climate will affect the biological transformation of inorganic mercury, an abundant pollutant that accumulates in arctic soil, into its neurotoxic form, methylmercury. Subsurface microbes catalyze the toxic conversion. Gu and his colleagues plan to measure and identify specific organic thiols, molecules thought to form a strong molecular bridge between microbes and mercury that influences the poisonous switch. What they find there could allow researchers to gauge potential health impacts of mercury and its global cycling as the planet warms.
Upscaling
One difficult challenge micromodelers of all forms face at some point is how to translate their findings up to environmentally relevant scales. “The problem is an obvious one,” says Tim Scheibe, a scientist at Pacific Northwest National Laboratory. “If we resolve things at a micron scale, how big a chunk of the earth can we actually simulate?” As of now, the answer to his question is about 2,000 cubic centimeters (the size of a laboratory column), “and that’s really pushing it,” Scheibe says. He uses supercomputer models to simulate processes such as contaminant transport at the pore scale, then attempts to ramp them up to larger scales.
While researchers will never be able to model kilometer-size sites at the pore scale (a feat Scheibe jokes would require “a million supercomputers”), they can get closer by employing efficient computing. To connect fundamental insights learned from pore-scale models up to meaningful magnitudes, Scheibe is working on what he calls “hybrid multiscale models.”
“The idea is that we directly connect the pore-scale model with a coarser model,” Scheibe says. The simulation then goes back and forth between scales, iteratively feeding information garnered from one scale into the other.
Scheibe also collaborates with researchers to incorporate data into pore-scale models that goes beyond just minerals and contaminants. Including information from the genome sequences of microbes known to live in the subsurface has informed Scheibe’s models of processes such as uranium bioremediation. Iron- and sulfur-reducing microbes such as native Geobacter species interact with natural minerals in the subsurface to create conditions under which uranium precipitates out of solution and becomes immobilized. With the aid of supercomputer simulations, Scheibe and colleagues have created models that account for the flexibility of microbes to regulate their metabolic processes in response to environmental conditions.
The fundamental insights EMSL scientists and users gain from zooming into the dynamic molecular events that make up the pore-scale world may help inform the decisions of engineers and policy makers.
“We can use this molecular information to understand where crops should be planted, how much sea level will rise, and where cities should be,” says Hess. “EMSL’s role is at the molecular level, yet it informs models at a much higher scale.”
Note : The above story is based on materials provided by Environmental Molecular Sciences Laboratory