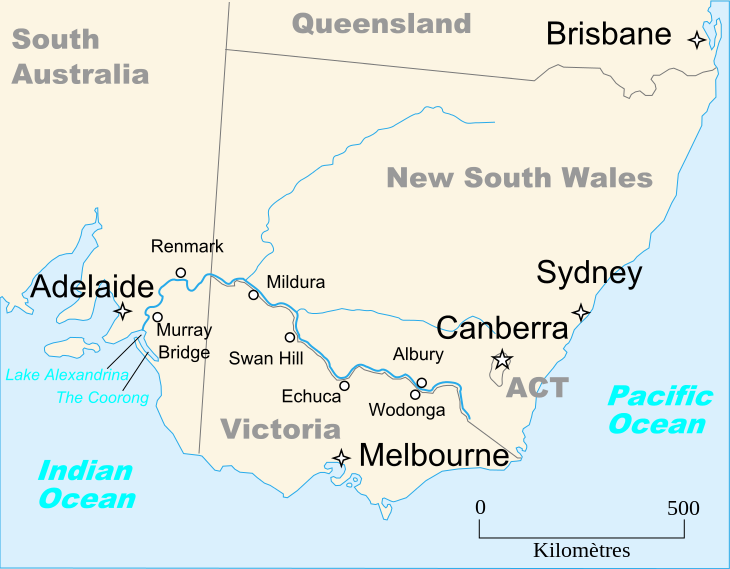
The Murray River (River Murray in South Australia) is Australia’s longest river. At 2,508 kilometres (1,558 mi) in length, the Murray rises in the Australian Alps, draining the western side of Australia’s highest mountains and, for most of its length, meanders across Australia’s inland plains, forming the border between the states of New South Wales and Victoria as it flows to the northwest, before turning south for its final 500 kilometres (310 mi) or so into South Australia, reaching the ocean at Lake Alexandrina.
The water of the Murray flows through several lakes that fluctuate in salinity (and were often fresh until recent decades) including Lake Alexandrina and The Coorong before emptying through the Murray Mouth into the southeastern portion of the Indian Ocean, often referenced on Australian maps as the Southern Ocean, near Goolwa. Despite discharging considerable volumes of water at times, particularly before the advent of largescale river regulation, the Mouth has always been comparatively small and shallow.
As of 2010, the Murray River system receives 58 percent of its natural flow. It is perhaps Australia’s most important irrigated region, and it is widely known as the food bowl of the nation.
Geography
The Murray River forms part of the 3,750 km (2,330 mi) long combined Murray-Darling river system which drains most of inland Victoria, New South Wales, and southern Queensland. Overall the catchment area is one seventh of Australia’s total land mass. The Murray carries only a small fraction of the water of comparably-sized rivers in other parts of the world, and with a great annual variability of its flow. In its natural state it has even been known to dry up completely during extreme droughts, although that is extremely rare, with only two or three instances of this occurring since official record keeping began.
The Murray River makes up much of the border between the Australian states of Victoria and New South Wales. Where it does, the border is the top of the bank of the southern side of the river (i.e., none of the river itself is actually in Victoria). This boundary definition can be ambiguous, since the river changes course, and some of the river banks have been modified.
West of the line of longitude 141°E, the river continues as the border between Victoria and South Australia for 3.6 km (2.2 mi), where this is the only stretch where a state border runs down the middle of the river. This was due to a miscalculation during the 1840s, when the border was originally surveyed. Past this point, the Murray River is entirely within the state of South Australia.
River life
The Murray River (and associated tributaries) support a variety of unique river life adapted to its vagaries. This includes a variety of native fish such as the famous Murray cod, trout cod, golden perch, Macquarie perch, silver perch, eel-tailed catfish, Australian smelt, and western carp gudgeon, and other aquatic species like the Murray short-necked turtle, Murray River crayfish, broad-clawed yabbies, and the large clawed Macrobrachium shrimp, as well as aquatic species more widely distributed through southeastern Australia such as common longnecked turtles, common yabbies, the small claw-less paratya shrimp, water rats, and platypus. The Murray River also supports fringing corridors and forests of the river red gum.
The health of the Murray River has declined significantly since European settlement, particularly due to river regulation, and much of its aquatic life including native fish are now declining, rare or endangered. Recent extreme droughts (2000 – 07) have put significant stress on river red gum forests, with mounting concern over their long term survival. The Murray has also flooded on occasion, the most significant of which was the flood of 1956, which inundated many towns on the lower Murray and which lasted for up to six months.
Introduced fish species such as carp, gambusia, weather loach, redfin perch, brown trout, and rainbow trout have also had serious negative effects on native fish, while carp have contributed to environmental degradation of the Murray River and tributaries by destroying aquatic plants and permanently raising turbidity. In some segments of the Murray, carp have become the only species found.
Note : The above story is based on materials provided by Wikipedia