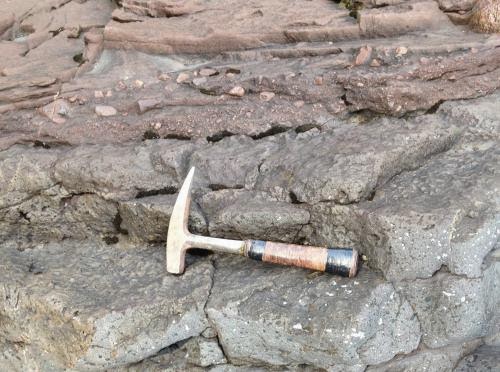
A team of biogeochemists at the University of California, Riverside, give us a nontraditional way of thinking about the earliest accumulation of oxygen in the atmosphere, arguably the most important biological event in Earth history.
A general consensus asserts that appreciable oxygen first accumulated in Earth’s atmosphere around 2.3 billion years ago during the so-called Great Oxidation Event (GOE). However, a new picture is emerging: Oxygen production by photosynthetic cyanobacteria may have initiated as early as 3 billion years ago, with oxygen concentrations in the atmosphere potentially rising and falling episodically over many hundreds of millions of years, reflecting the balance between its varying photosynthetic production and its consumption through reaction with reduced compounds such as hydrogen gas.
“There is a growing body of data that points to oxygen production and accumulation in the ocean and atmosphere long before the GOE,” said Timothy W. Lyons, a professor of biogeochemistry in the Department of Earth Sciences and the lead author of the comprehensive synthesis of more than a decade’s worth of study within and outside his research group.
Lyons and his coauthors, Christopher T. Reinhard and Noah J. Planavsky, both former UCR graduate students, note that once oxygen finally established a strong foothold in the atmosphere starting about 2.3 billion years ago it likely rose to high concentrations, potentially even levels like those seen today. Then, for reasons not well understood, the bottom fell out, oxygen plummeted to a tiny fraction of today’s level, and the ocean remained mostly oxygen free for more than a billion years.
The paper appears in Nature on Feb. 19.
“This period of extended low oxygen spanning from roughly 2 to less than 1 billion years ago was a time of remarkable chemical stability in the ocean and atmosphere,” Lyons said.
His research team envisions a series of interacting processes, or feedbacks, that maintained oxygen at very low levels principally by modulating the availability of life-sustaining nutrients in the ocean and thus oxygen-producing photosynthetic activity.
“We suggest that oxygen was much lower than previously thought during this important middle chapter in Earth history, which likely explains the low abundances and diversity of eukaryotic organisms and the absence of animals,” Lyons said.
The late Proterozoic—the time period beginning less than a billion years ago following this remarkable chapter of sustained low levels of oxygen—was strikingly different, marked by extreme climatic events manifest in global-scale glaciation, indications of at least intervals of modern-like oxygen abundances, and the emergence and diversification of the earliest animals. Lyons notes that the factors controlling the rise of animals are under close scrutiny, including challenges to the long-held view that a major rise in atmospheric oxygen concentrations triggered the event.
“Despite the new ideas about animal origins, we suspect that oxygen played a major if not dominant role in the timing of that rise and, in particular, in the subsequent emergence of complex ecologies for animal life on and within the sediment, predator-prey relationships, and large bodies” said Lyons. “But, again, feedbacks always rule the day. Environmental change drives evolution, and steps in the progression of life change the environment.”
No single factor is likely to be the whole story, and there is much more to be written in the tale. Lyons and coauthors, along with research groups from around world over, are focusing current efforts on the timing and drivers of oxygenation in the late Proterozoic, favoring a combination of global-scale mountain building, evolutionary controls on the way carbon is cycled in the biosphere, and concomitant climate events.
“We are faced with a lot of chicken-and-egg questions when it comes to unraveling the timing and sequence of oxygenation of the ocean and atmosphere,” Lyons said. “But now, armed with new and better data, more sophisticated numerical simulations, and highly integrated investigations in the lab and the field, Earth’s oxygenation history seems much longer and more dynamic than envisioned before, and we are getting closer to understanding the mechanisms behind such change.”
Note : The above story is based on materials provided by University of California – Riverside